1. Background
Transparent materials are widely used in daily life due to their unique physical properties of high transparency. Transparent ceramics can be applied harsh environment due to various advantages such as high hardness and strength, excellent physical and chemical stability. With the rapid development of science and technology, the requirements of functional materials are ever-increasing. Meanwhile, transparent functional ceramics need novel properties, for instance, ferroelectricity, scintillation and laser characteristics.
Potassium-sodium niobate (KNN) ceramics are on kind of environmentally friendly lead-free piezoelectric ceramics, possessing superiority including high piezoelectric coefficient, high Curie temperature and high defect tolerance. And they are easy to realize physical coupling (e.g. electromechanical, magnetoelectric, electro-optic). Recently, A/Profs. Cong Lin & Xiao Wu's group prepared a variety of KNN-based multifunctional transparent ceramics via pressureless sintering, exhibiting optical transparency, piezoelectricity, ferroelectric energy-storage feature, electro-optic effect, photoluminescence and photochromism. The materials have great potential in the applications of transparent capacitors, optical-electrical integrators, optical switches and fluorescent temperature sensors.
2. Research Achievements
2.1 Photos of various KNN transparent ceramics
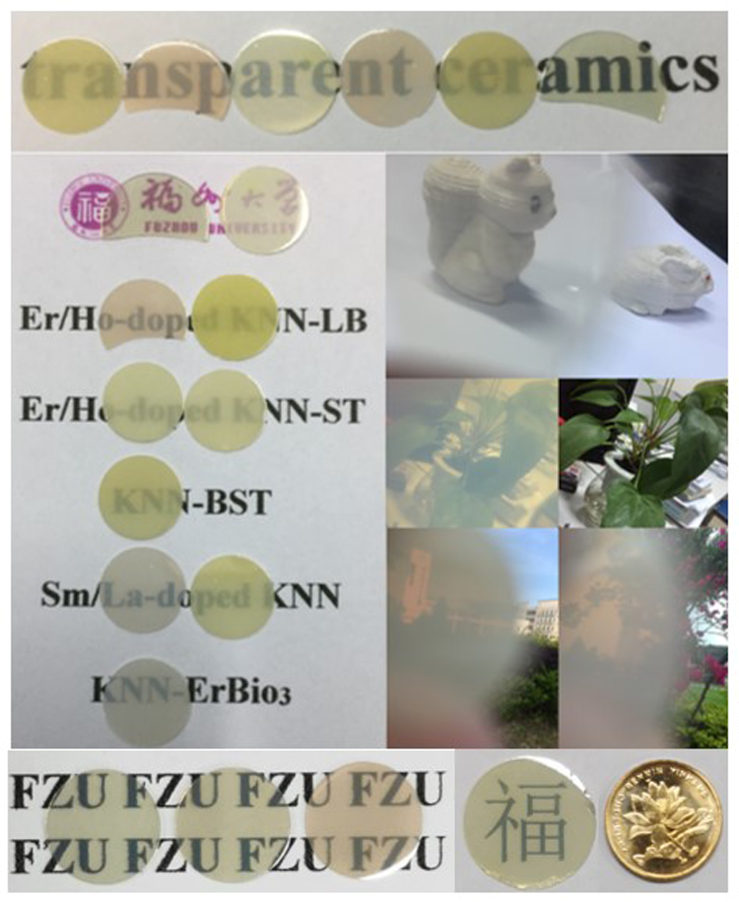
Fig. 1 Various KNN-based transparent ceramics via pressureless sintering (with the thicknesses of 0.3-0.4 mm).
2.2 Electro-optic effect of KNN transparent ceramics
Electro-optic (EO) effect is a phenomenon in which the optical properties (such as refractive index) of a material can be changed under an external electric field. Through modification of Li/Bi and doping of rare-earth Er, the cubic phase structures KNN-LB-x and Er-KNN-LB-x transparent ceramics have strong EO effect with linear EO coefficients of 180-200 pm/V, far higher than other commercial EO materials such as LiNbO3, LiTaO3 and KDP single crystals and comparable to that of lead-based PMN-PT single crystals. Considering the environmental friendliness of lead-free material and low cost of preparing transparent ceramics without special sintering, the KNN-based transparent ceramics can be applied in EO devices (e.g. light attenuators and electrical-controlled light switches).
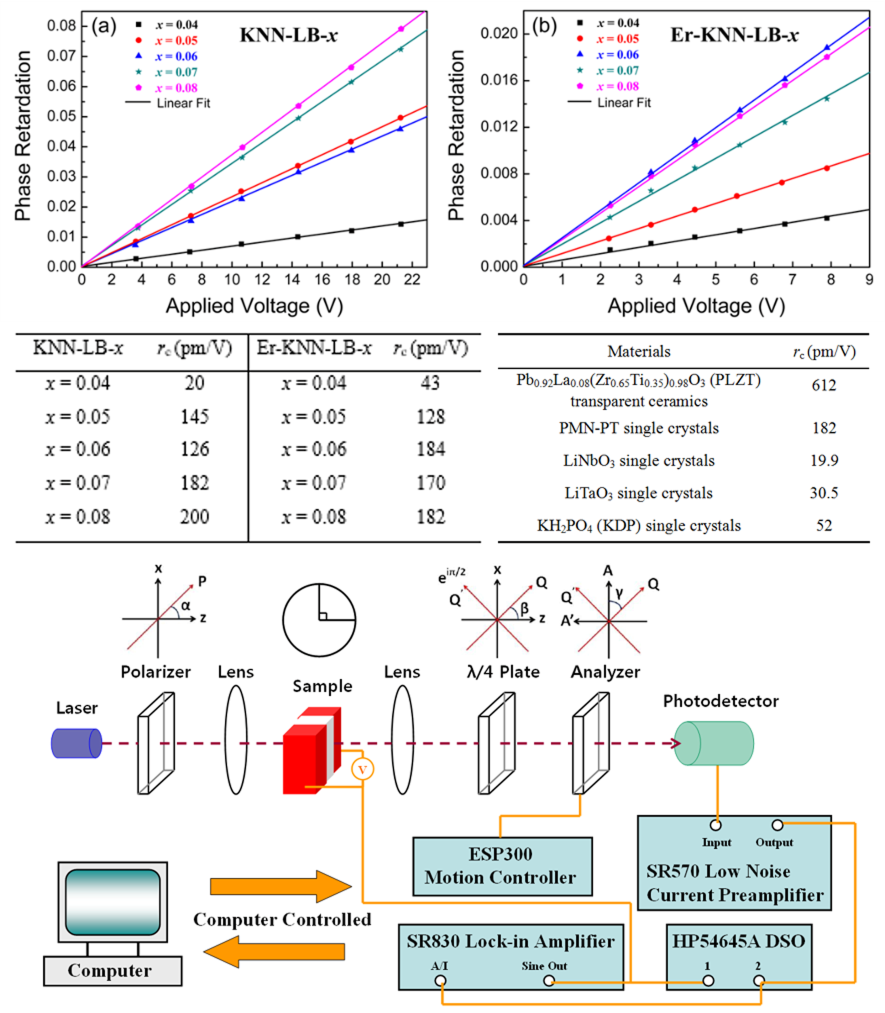
Fig. 2 Comparison of linear EO coefficients of KNN-LB-x and Er-KNN-LB-x transparent ceramics and other commercial EO single crystals. Schematic testing devices for measuring EO coefficients (J. Alloys Comp., 706, 156-163, 2017).
2.3 Photochromism of KNN transparent ceramics
Photochromism means that an object produces color changes under light irradiation, and the color can return to the initial state by heating or light stimulation. Inorganic photochromic materials exhibit excellent optical, electrical and magnetic properties and are widely used in optical switches, data storages, displays, temperature recording media, optical labeling and other fields. During the process of discoloration, the chemical/physical behavior simultaneously changes, including luminescence intensity, light transmittance, oxidation-reduction potential, dielectric constant and magnetism. Rare-earth doped KNN-based transparent ceramics can produce obvious photochromism, which can further induce reversible modulations of both transmittance and luminescence intensity.
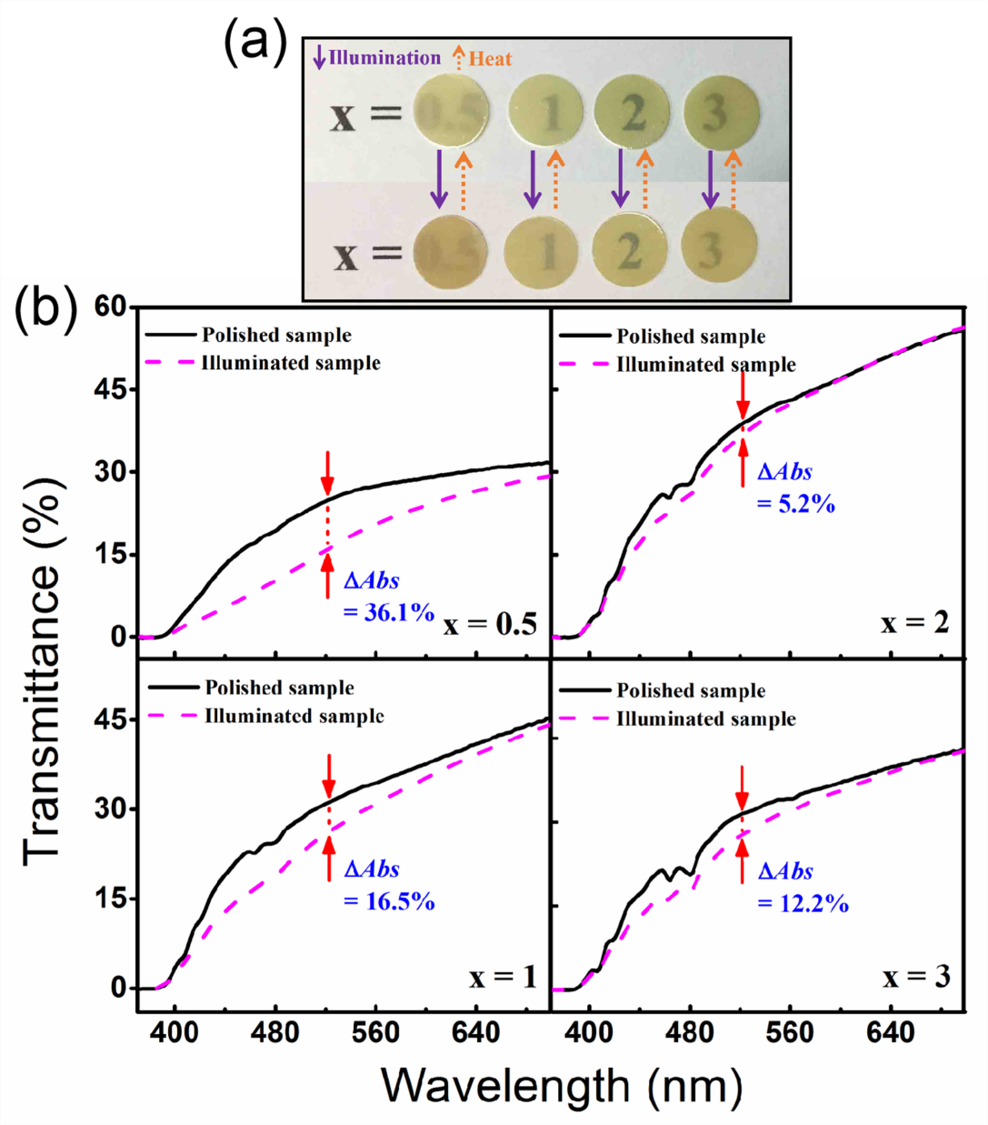
Fig. 3 (a) Photographs of the KNN-Sm-x transparent ceramics under illumination from a xenon lamp (200-1100 nm, 300 W, 5 min) and after the thermal stimulus (200ºC, 5 min). (b) The optical transmittance spectra of the KNN-Sm-x transparent ceramics before and after illumination (J. Mater. Chem. A, 7, 19374-19384, 2019).
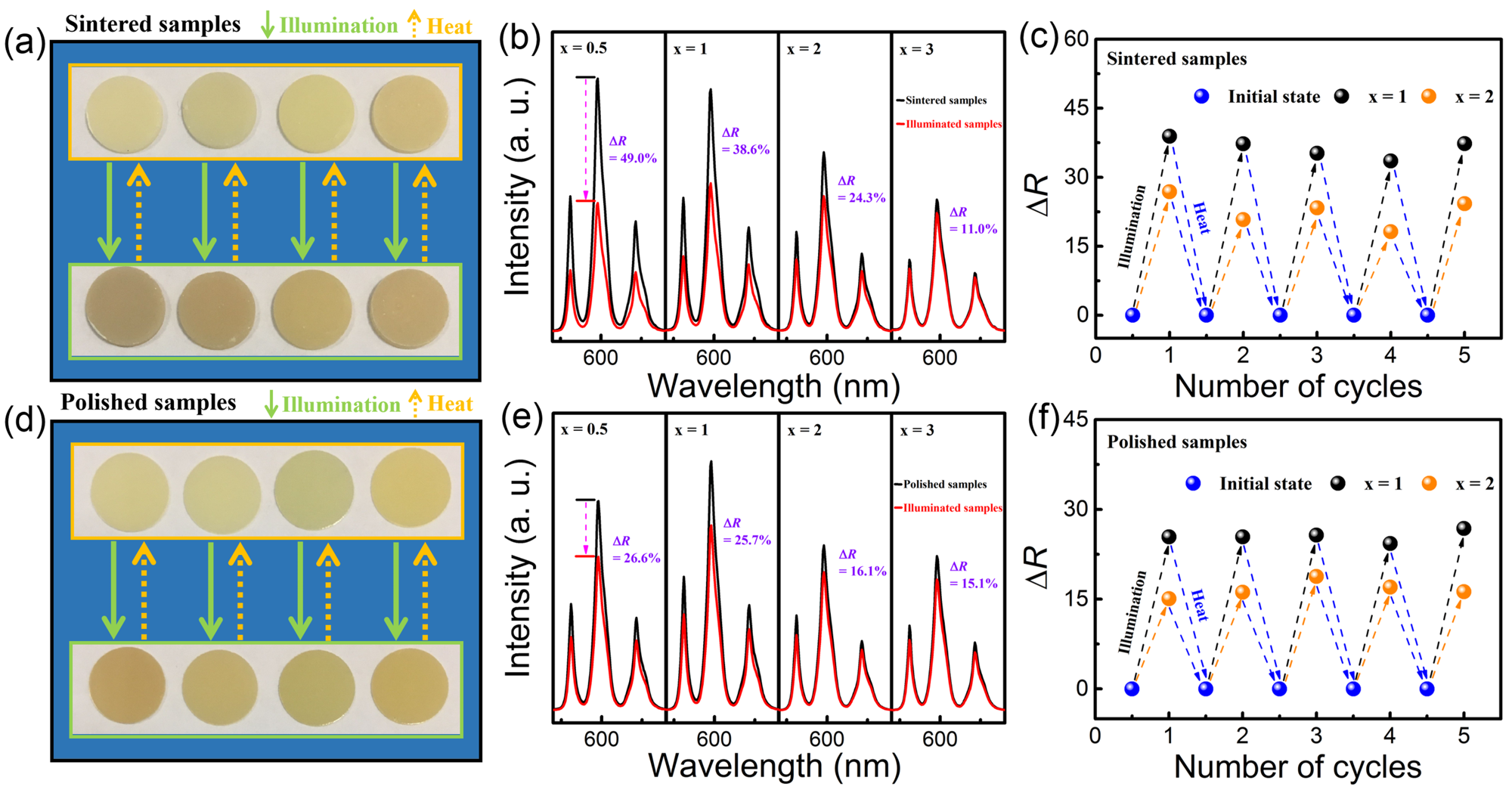
Fig. 4 (a and d) Photographs, (b and e) photoluminescence spectra and (c and f) luminescence switching ratio (DR) monitoring at 597 nm emission intensity of the fresh sintered and polished KNN-Sm-x ceramics, by alternating illumination and thermal stimulus (J. Mater. Chem. A, 7, 19374-19384, 2019).
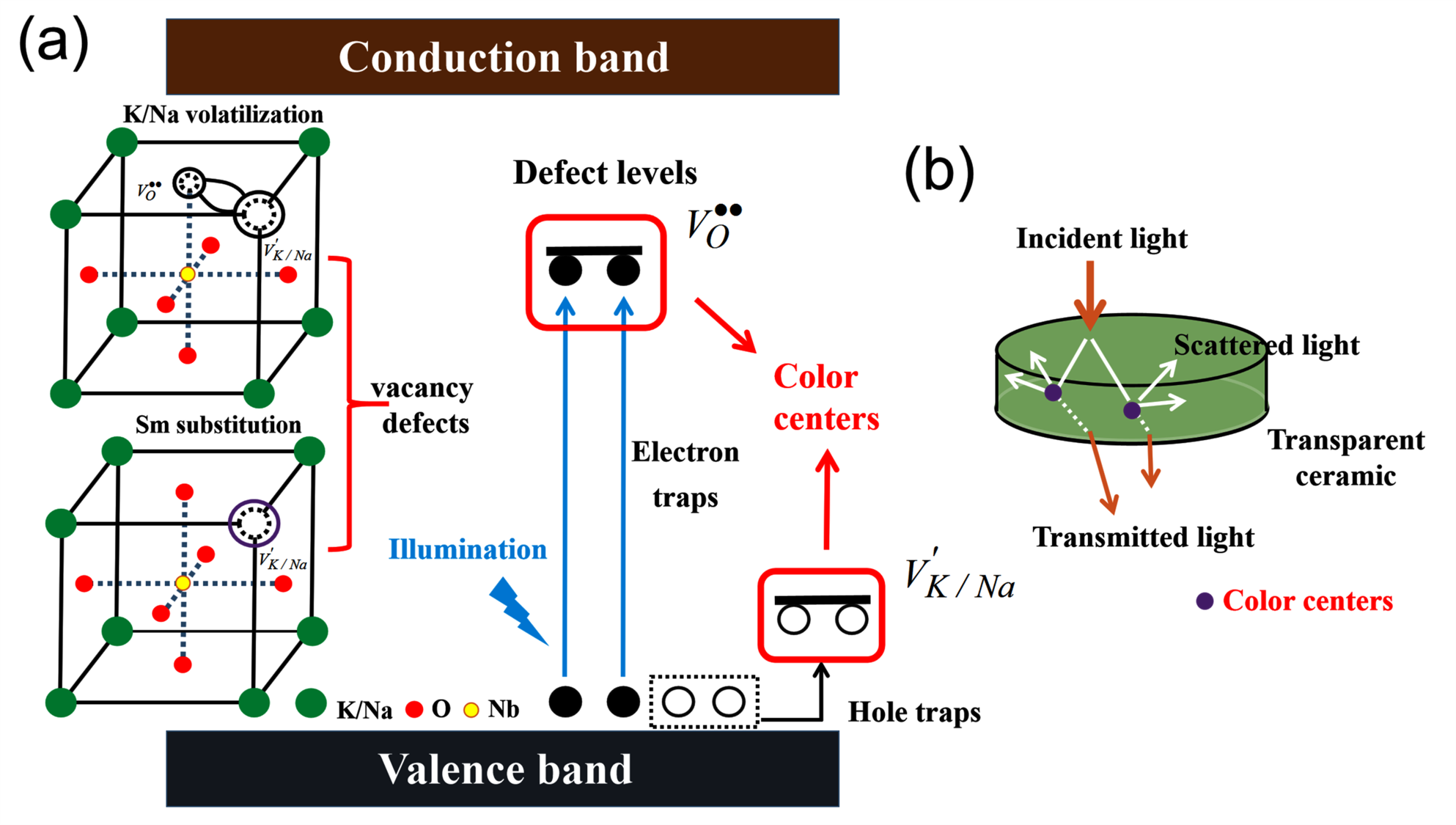
Fig. 5 (a) The diagram of the photochromic mechanism in the KNN-Sm-x ceramics and (b) the modulating mechanism of optical transmittance of the KNN-Sm-x ceramics (J. Mater. Chem. A, 7, 19374-19384, 2019).
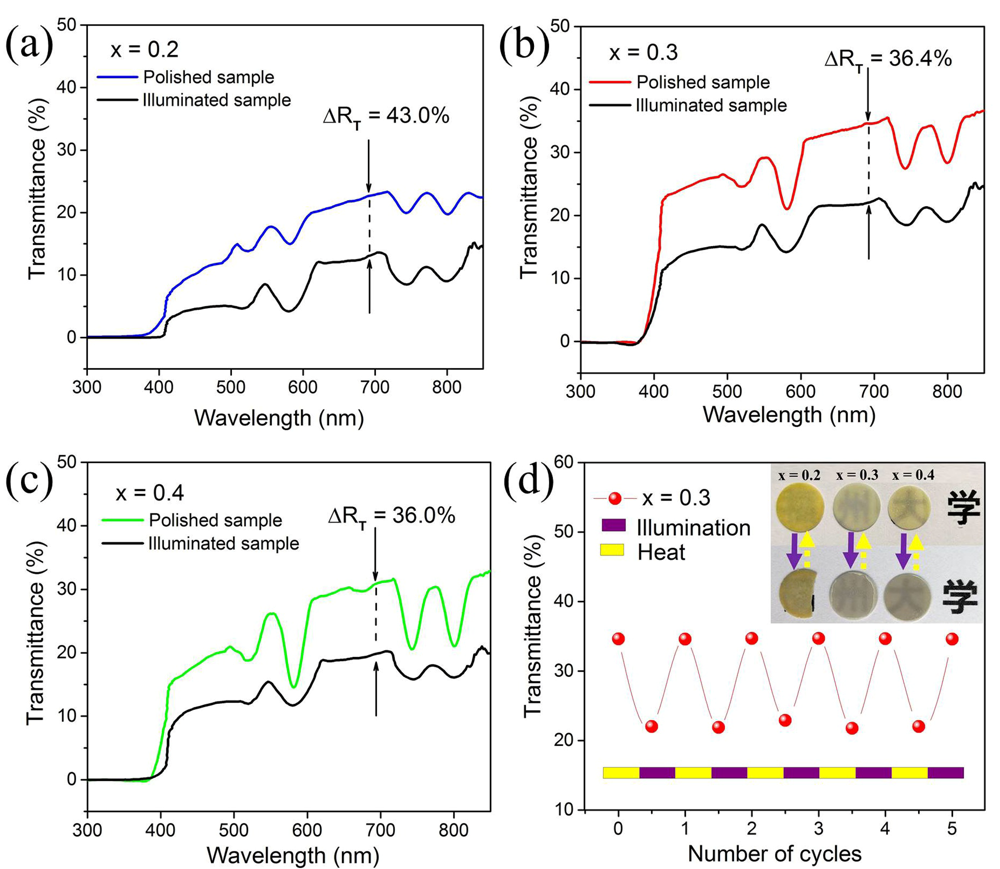
Fig. 6 (a-c) The optical transmittance spectra of the xNd-KNN translucent ceramics before and after 365 nm illumination and (d) photographs of the ceramics and the transmittance switching for 5 cycles of 0.3Nd-KNN under illumination with an ultraviolet lamp (365 nm, 5 W) for 20 s and after thermal stimulus (210ºC, 10 min) (J. Mater. Chem. C, 8, 2343-2352, 2020).
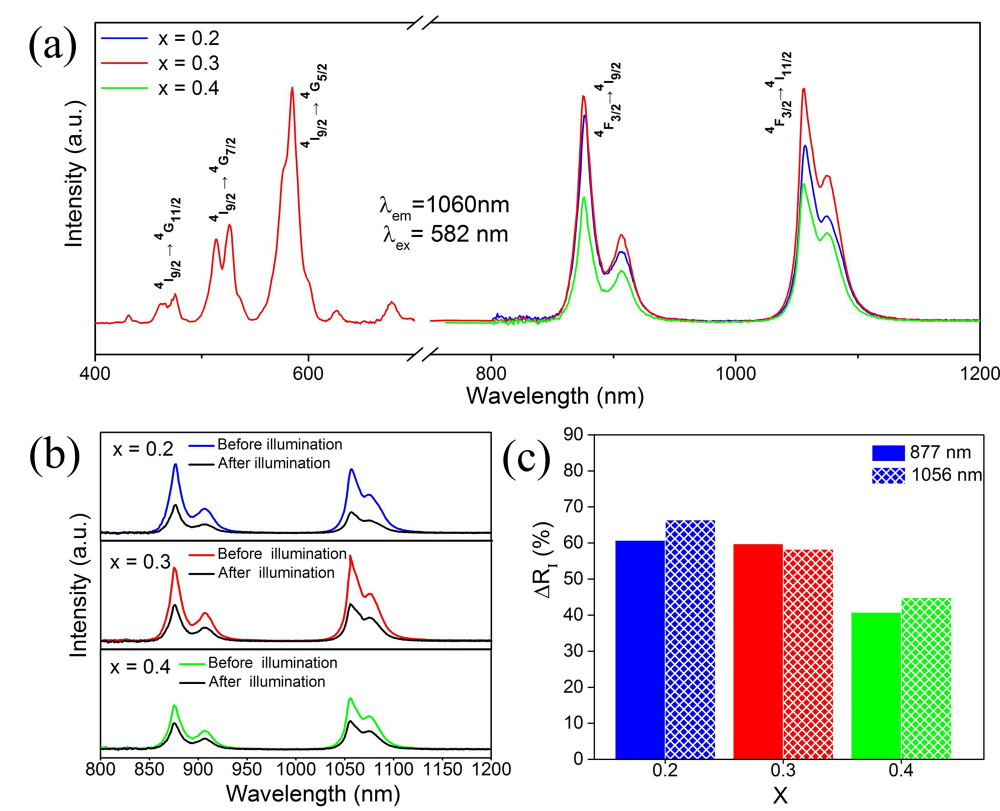
Fig. 7 (a) Down-shifting photoluminescence excitation and emission spectra under 582 nm excitation, (b) emission spectra before and after 365 nm illumination, (c) ΔRI values monitored at 877 nm and 1056 nm emissions of the xNd-KNN ceramics(J. Mater. Chem. C, 8, 2343-2352, 2020).
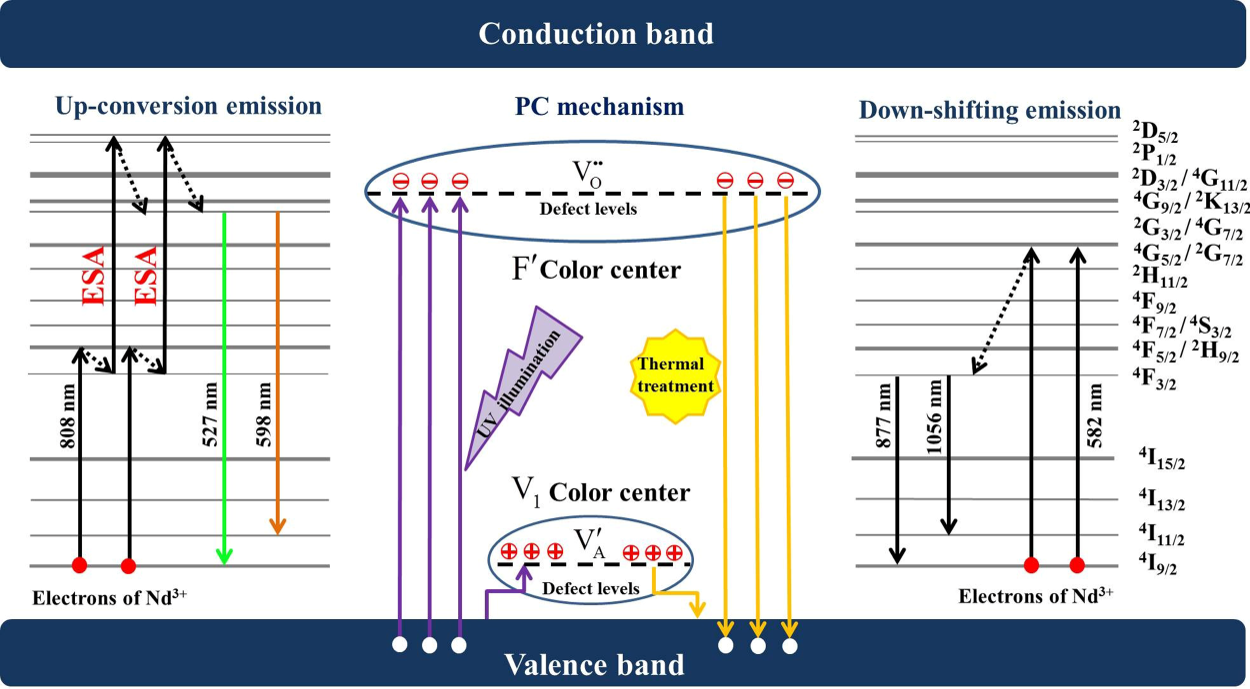
Fig. 8 The energy level diagram of Nd3+ and the photochromic modulation mechanism in the xNd-KNN ceramics (J. Mater. Chem. C, 8, 2343-2352, 2020).
2.4 Ferroelectric energy-storage properties of KNN transparent ceramics
KNN-based ceramics with excellent light transmittance and good energy-storage characteristics can provide more material choices for energy-storage transparent electronics. The Li/Bi modified and Er doped Er-KNN-LB-0.05 transparent ceramics were prepared, exhibiting highest light transmittance of over 80% in the visible region. Meanwhile, good ferroelectric energy-storage characteristics were obtained at a high electric field of 260 kV/cm, and the charged and discharge energy densities were 5.87 J/cm3 and 1.96 J/cm3 respectively.
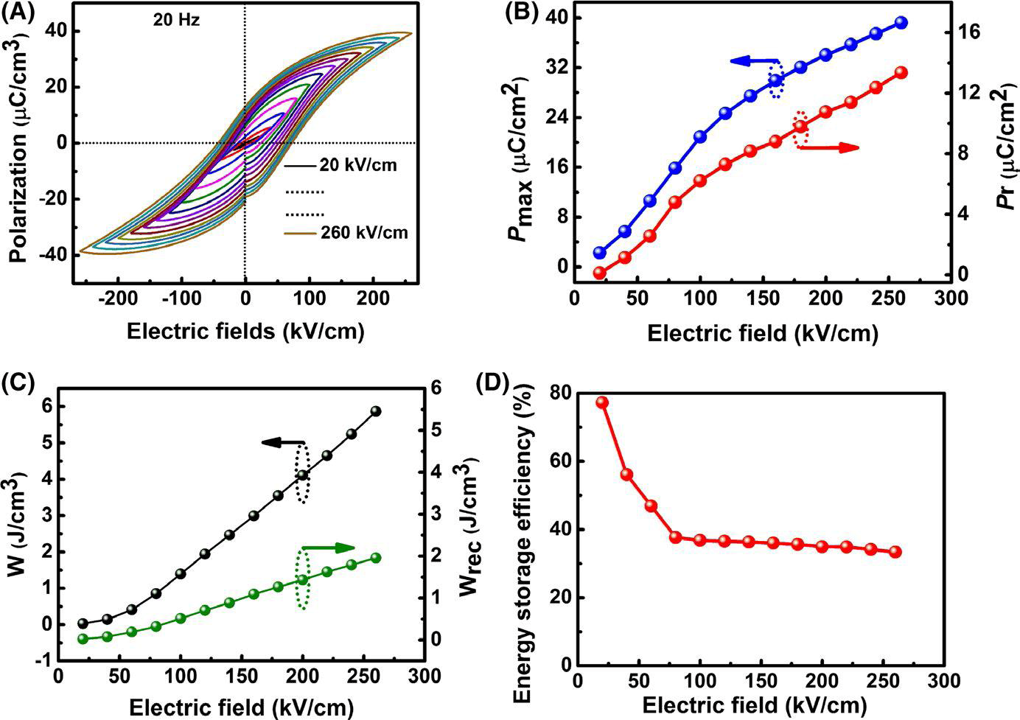
Fig. 9 The P‐E loops of the Er-KNN-LB-0.05 transparent ceramic and energy-storage properties of the material (J. Am. Ceram. Soc., 102, 6732-6740, 2019).
2.5 Optical temperature sensing performance of KNN transparent ceramics
Ho-doped KNN-SrTiO3 (xHo-KNNST) ceramics possess high optical transmittance and easy-modulated up-conversion luminescence characteristics (with stable emission color within 200°C as well as sensitive and regulated emission colors with temperature above 200°C). In addition, the ceramics also exhibit excellent optical temperature sensing characteristics (with the sensitivity up to 0.0096/K), higher than other rare-earth doped ferroelectric ceramics and many non-ferroelectric luminescent materials. The xHo-KNNST ceramics have application potentials in color-stable optical displays, transparent solid-state lasers and optical temperature sensors.
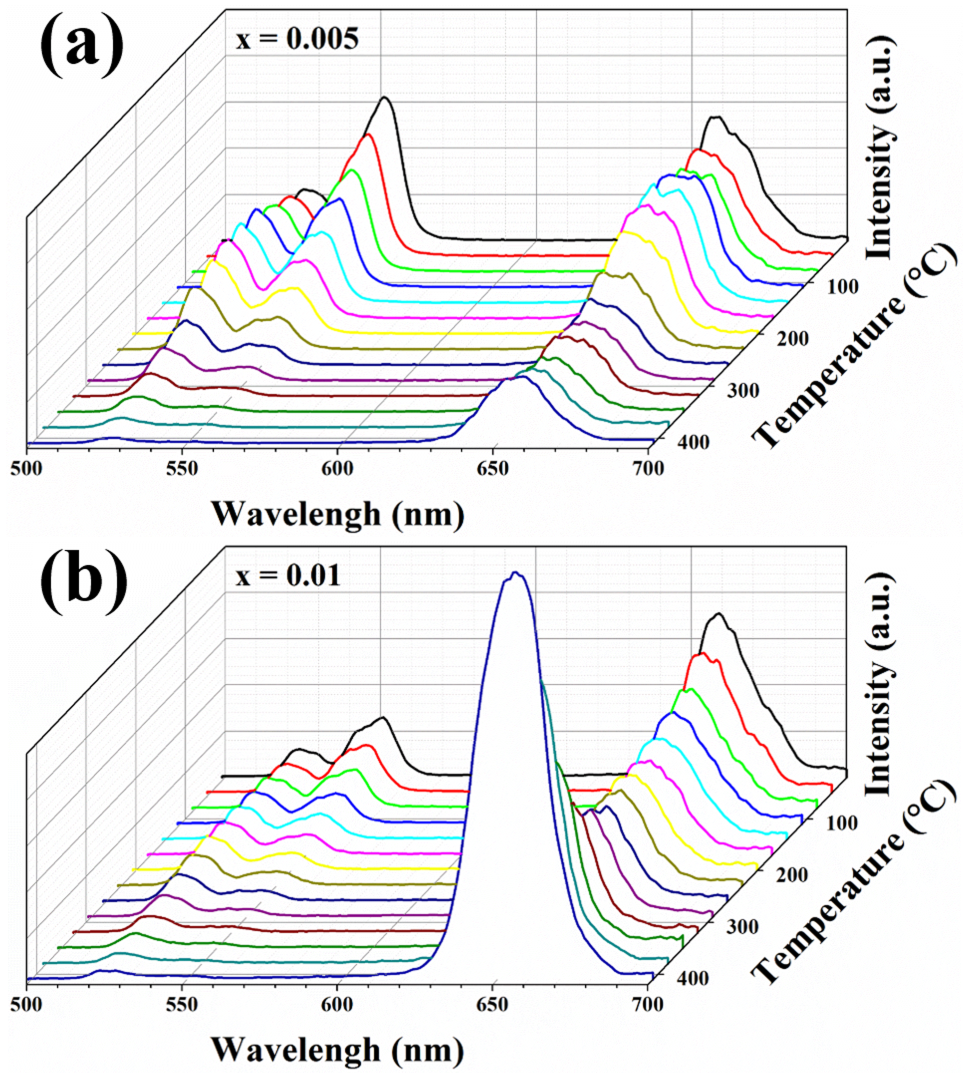
Fig. 10 Temperature‐dependent up‐conversion emission spectra of the xHo‐KNNST ceramics with (A) x = 0.005 and (B) x = 0.01 ranging from room temperature to 410ºC (J. Am. Ceram. Soc., 102, 1249-1258, 2019).
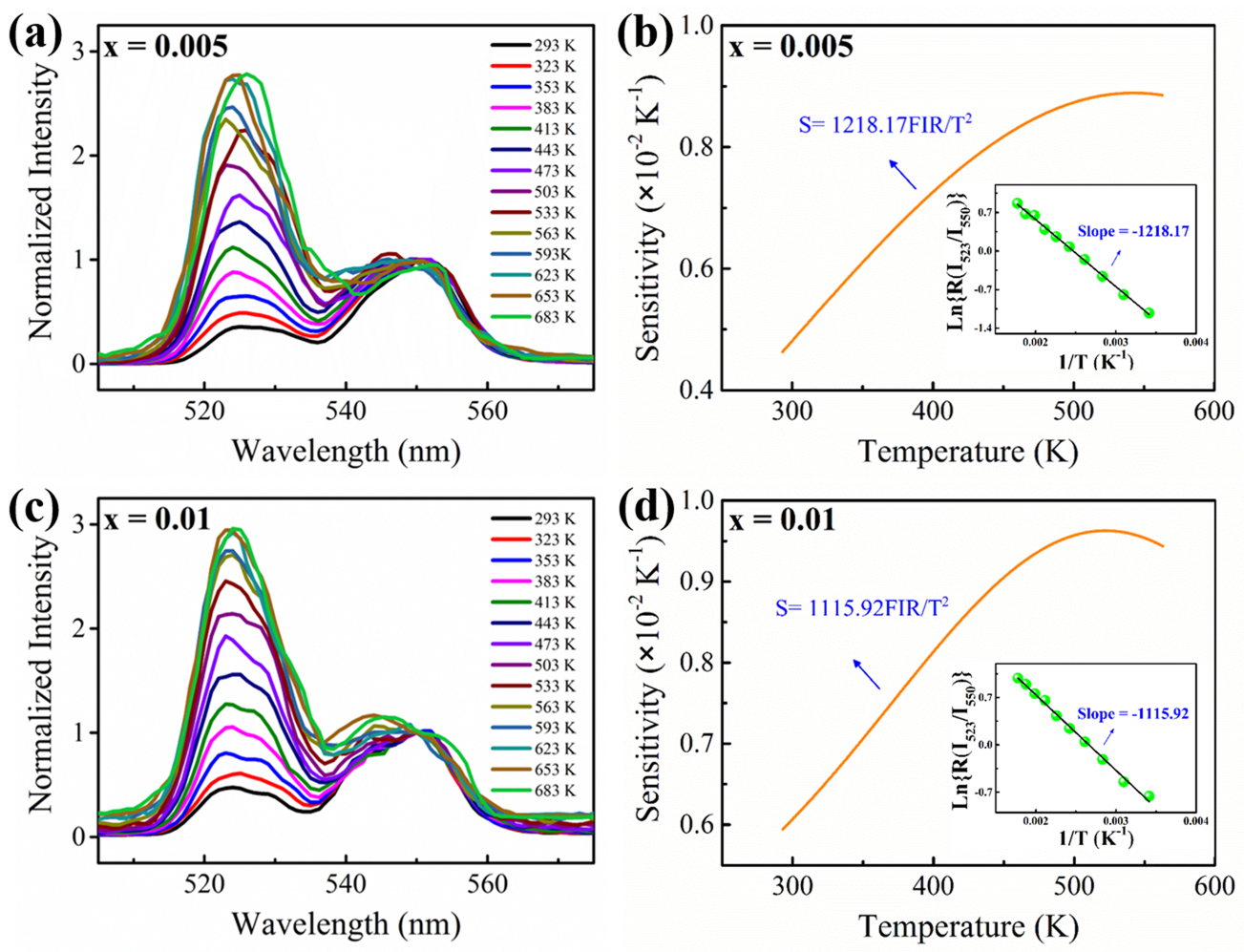
Fig. 11 Variation in up‐conversion green emission spectra with temperatures in the range 293‐683 K for the xHo‐KNNST ceramics with (A) x = 0.005 and (C) x = 0.01, sensitivity as a function of temperature for (B) x = 0.005 and (D) x = 0.01. The insets of (B) and (D) are lognormal plots of the FIR values as a function of inverse absolute temperature for x = 0.005 and x = 0.01, respectively (J. Am. Ceram. Soc., 102, 1249-1258, 2019).
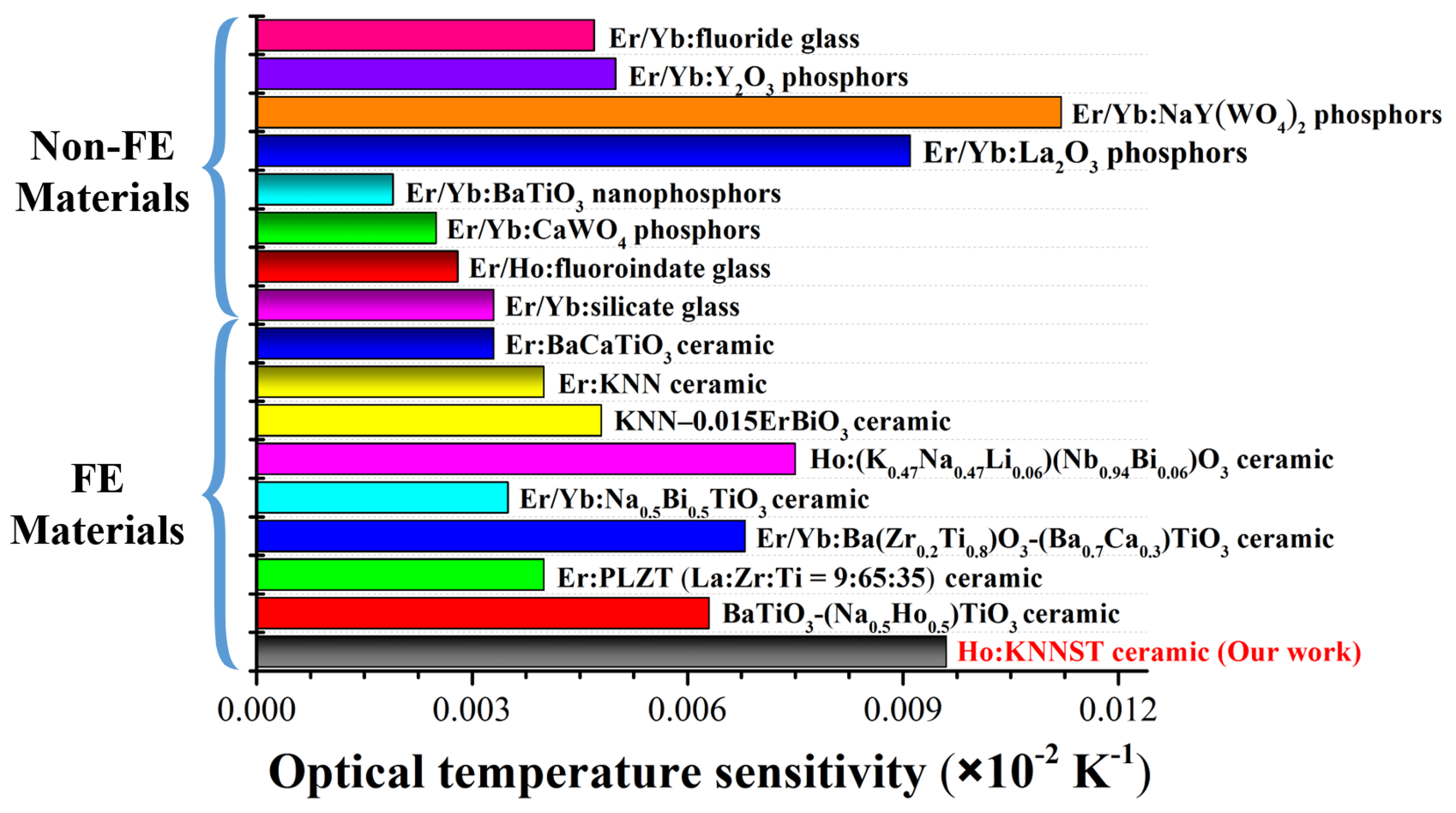
Fig. 12 The optical temperature sensing properties based on up‐conversion emission of main RE‐doped ferroelectric (FE) ceramics and RE‐doped/codoped nonferroelectric (Non‐FE) materials (including glasses, phosphors, and nanophosphors) (J. Am. Ceram. Soc., 102, 1249-1258, 2019).
2.6 Electric field regulated luminescence KNN transparent ceramics
Based on different photoluminescence processes, there are two modes of photoluminescence, down-shifting photoluminescence (DCPL) and up-conversion photoluminescence (UCPL). And materials possessing excellent dual-mode luminescence characteristics are worth studying. Common methods used to enhance or adjust the photoluminescence response include optimizing the preparation process, changing the concentration of emission centers, changing the composition of matrix, etc. However, these chemical methods are essentially irreversible and non in-situ processes, which are not conducive to revealing the dynamic process and practical application of photoluminescence. Therefore, it is necessary to explore physical routes to realize in-situ and real-time regulation of photoluminescence on the same material. Ferroelectrics have spontaneous polarization and the inner dipoles can be switched and the lattice symmetry can slightly change under the external electric field. For the Er-doped KNN transparent ceramics, the dual-mode DCPL and UCPL intensities were effectively regulated by an in-situ, real-time, reversible and dynamic manner through dipole-switching and change of local structure around Er3+.
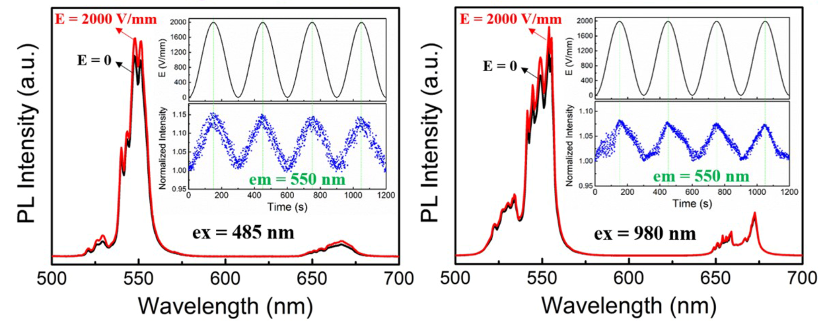
Fig. 13 Dual mode DCPL and UCPL spectra of Er-doped KNN transparent ceramic slices under an in-situ electric field. The insets show the real-time and reversible regulations of luminescence intensity at 550 nm under sinusoidal AC electric fields (J. Mater. Chem. C, 7, 7885-7892, 2019).
2.7 DFT calculations in the KNN system
For all kinds of rare-earth doped KNN transparent ceramics, effective modulation of luminescence behavior can be realized based on photochromic reaction or applied electric field. The change tendency of vacancy defects within KNN and the influence of electronic structure and lattice environment on luminescence behavior of emission centers in KNN should be further explored by theoretical calculations, in order to reveal the relationship between photoluminescence/photochromism and crystal structure. Density functional theory (DFT) can help simulate the substitution behavior, vacancy defect concentration and energy band structure of materials, considered as an effective method for elucidating the mechanism of ferroelectric spontaneous polarization, energetics and phase diagrams.
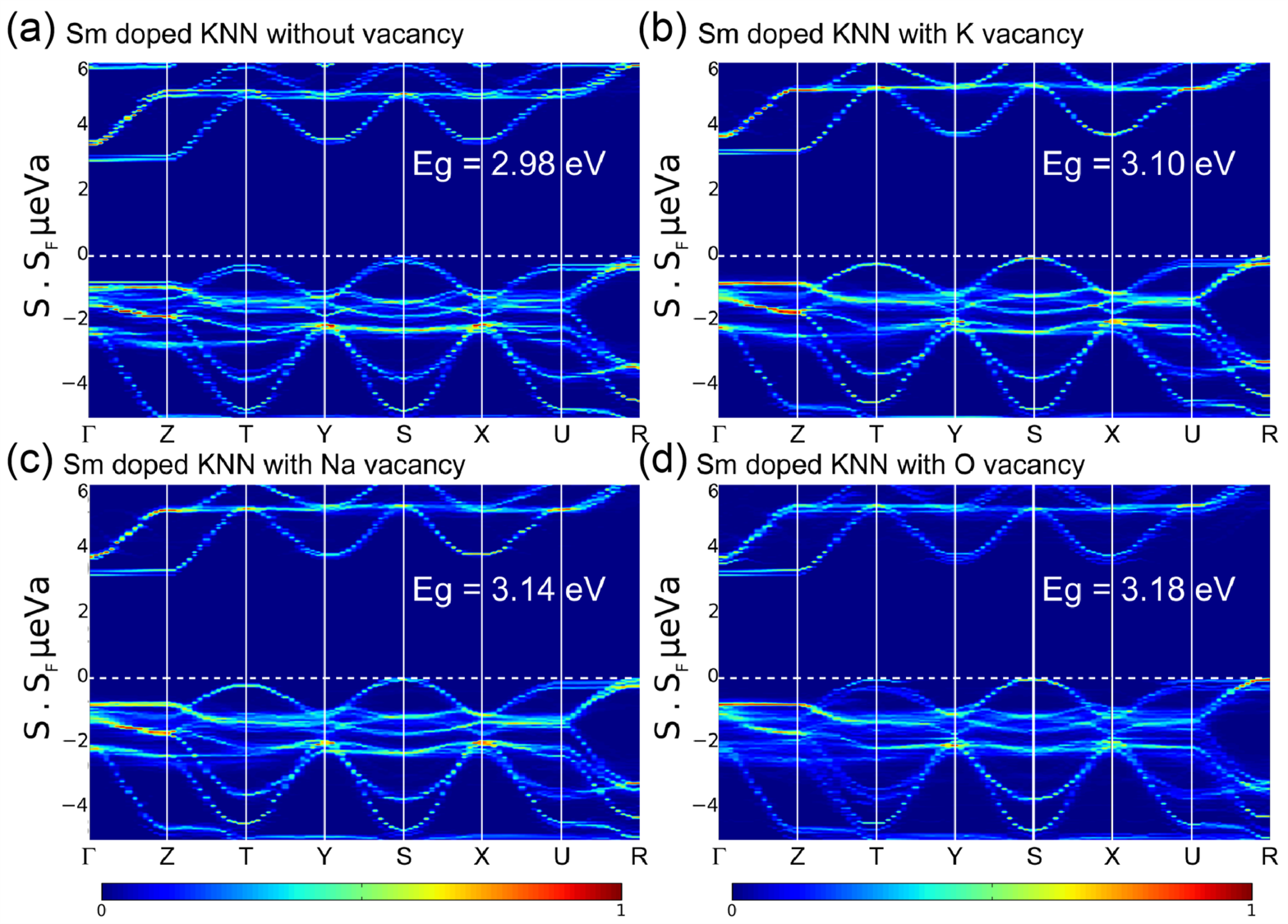
Fig. 14 The unfolded primitive cell representation of band structures of four kinds of KNN-Sm-2. (a) Without any vacancy, (b) with the K vacancy, (c) with the Na vacancy and (d) with the O vacancy (J. Mater. Chem. A, 7, 19374-19384, 2019).
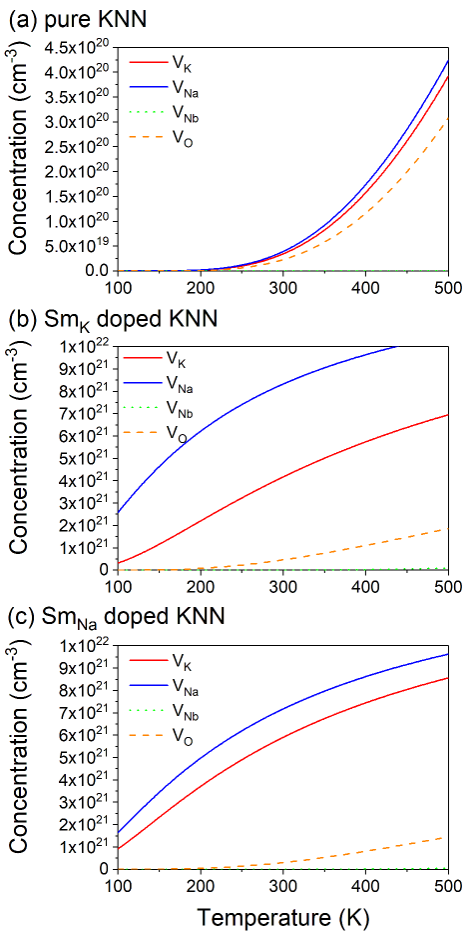
Fig. 15 Different types of vacancy concentrations as a function of temperature for (a) pure KNN and Sm-doped KNN ((b) SmK doped KNN and (c) SmNa doped KNN) in different replacement environments (J. Mater. Chem. A, 7, 19374-19384, 2019).
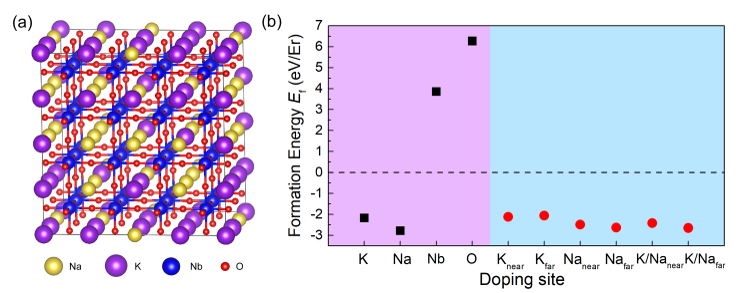
Fig. 16 (a) The SQS structure of the KNN 4 × 4 × 3 supercell including 240 atoms, (b) the formation energy of Er-doped KNN with different Er-doping sites (J. Mater. Chem. C, 7, 7885-7892, 2019).
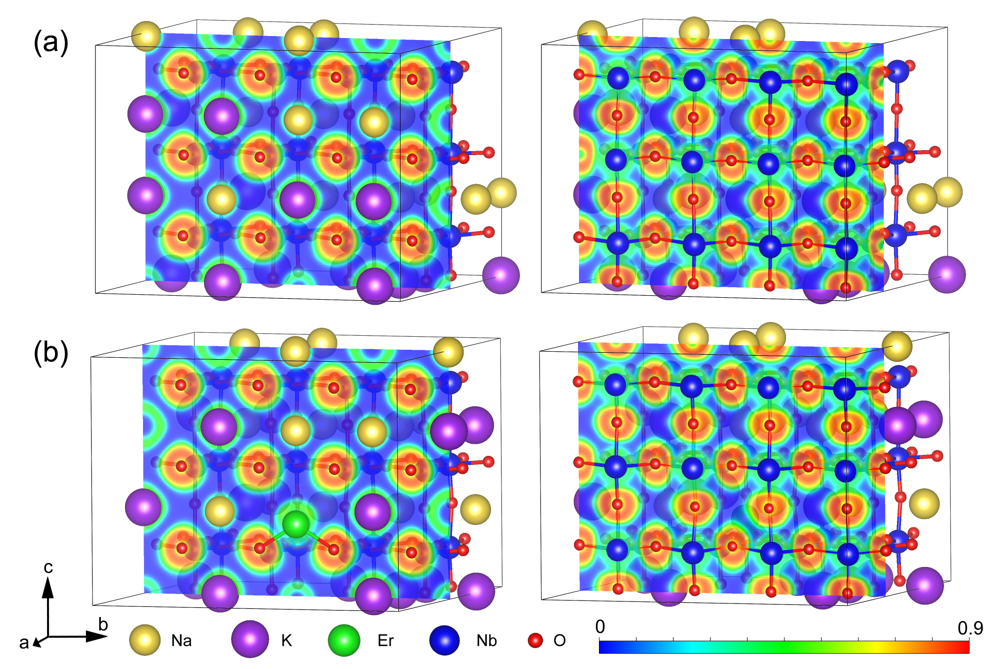
Fig. 17 Plots of electron localization function in the (100) plane of (a) pure KNN and (b) Er-doped KNN (J. Mater. Chem. C, 7, 7885-7892, 2019).
Links:
(1)https://pubs.rsc.org/en/content/articlelanding/2020/tc/c9tc05899k/unauth#!divAbstract (J. Mater. Chem. C, 8, 2343-2352, 2020).
(2)https://pubs.rsc.org/ko/content/articlelanding/2019/ta/c9ta05936a#!divAbstract(J. Mater. Chem. A, 7, 19374-19384, 2019).
(3)https://ceramics.onlinelibrary.wiley.com/doi/abs/10.1111/jace.16336 (J. Am. Ceram. Soc., 102, 1249-1258, 2019)
(4)https://ceramics.onlinelibrary.wiley.com/doi/abs/10.1111/jace.16534 (J. Am. Ceram. Soc., 102, 6732-6740, 2019)
(5)https://pubs.rsc.org/en/content/articlelanding/2019/tc/c9tc01356c/unauth#!divAbstract (J. Mater. Chem. C, 7, 7885-7892, 2019).
(6)https://www.sciencedirect.com/science/article/abs/pii/S0925838816338920(J. Alloys Comp., 706, 156-163, 2017).